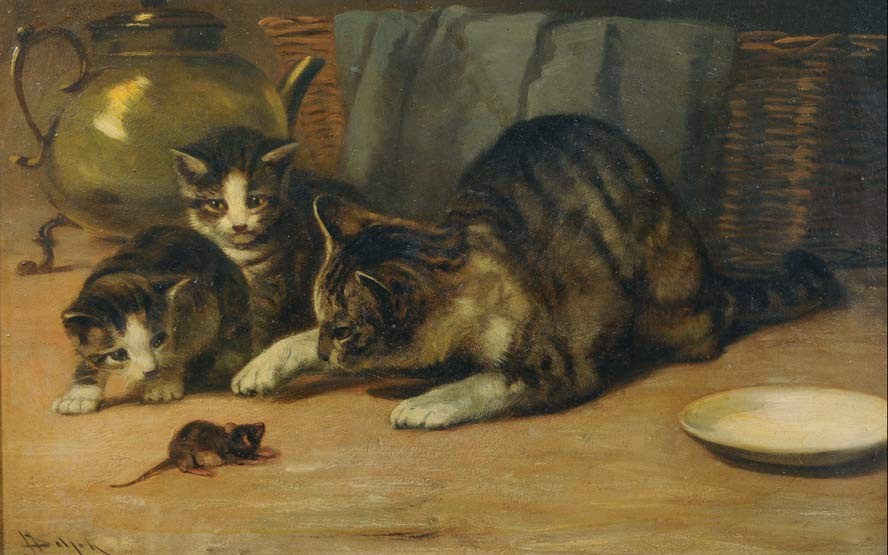
If 6 cats can kill 6 rats in 6 minutes, how many will be needed to kill 100 rats in 50 minutes?
It’s easy enough to work out that the answer is 12, but consider what this means. “When we come to trace the history of this sanguinary scene through all its horrid details, we find that at the end of 48 minutes 96 rats are dead, and that there remain 4 live rats and 2 minutes to kill them in,” observed Lewis Carroll in the Monthly Packet in February 1880. “The question is, can this be done?”
Consider the original statement: 6 cats can kill 6 rats in 6 minutes. What can this actually mean? Carroll counts at least four possibilities:
A. “All 6 cats are needed to kill a rat; and this they do in one minute, the other rats standing meekly by, waiting for their turn.”
B. “3 cats are needed to kill a rat, and they do it in 2 minutes.”
C. “2 cats are needed, and they do it in 3 minutes.”
D. “Each cat kills a rat all by itself, and takes 6 minutes to do it.”
Now try to apply these to our conclusion that 12 cats can kill 100 rats in 50 minutes. Cases A and B work out, but Case C can work only if we understand that fractional deaths are possible: that 2 cats could kill two-thirds of a rat in 2 minutes. Similarly, Case D works only if a cat can kill one-third of a rat in 2 minutes.
The only way to resolve this absurdity, it seems, is to supply extra cats. “In case C less than 2 extra cats would be of no use. If 2 were supplied, and if they began killing their 4 rats at the beginning of the time, they would finish them in 12 minutes, and have 36 minutes to spare, during which they might weep, like Alexander, because there were not 12 more rats to kill. In case D, one extra cat would suffice; it would kill its 4 rats in 24 minutes, and have 24 minutes to spare, during which it could have killed another 4. But in neither case could any use be made of the last 2 minutes, except to half-kill rats — a barbarity we need not take into consideration.”
“To sum up our results: If the 6 cats kill the 6 rats by method A or B, the answer is ’12’; if by method C, ’14’; if by method D, ’13’.”
(Another problem: “If a cat can kill a rat in a minute, how long would it be killing 60,000 rats? Ah, how long, indeed! My private opinion is that the rats would kill the cat.”)